|
 |
Gas Discharge
Tubes - Introduction
|
A gas discharge tube is a bulb or
tube (usually glass), with two (or more) electrodes inserted into it,
that has been evacuated and filled with a gas or gas mixture usually at
somewhat less than atmospheric pressure. All such tubes have the
property that, as the voltage applied across the electrodes is
increased, there comes a point called the 'strike voltage' or
'breakdown voltage', at which any localised ionisation of the gas will
initiate an avalanche process that spreads through the tube. The
voltage at which breakdown occurs depends on the composition and
pressure of the gas mixture and the strength of the applied electric
field according to a relationship known as 'Paschen's law'. Somewhat
surprisingly, the breakdown voltage does not simply increase with
electrode spacing, but follows a bathtub curve, i.e., there is a range
of spacings for which the breakdown voltage actually increases as the
electrodes get closer together. The reason for that is that breakdown
depends on the probability of collisions between electrons and atoms,
and there is a regime in which shortening the inter-electrode distance
makes the collision probability too low to initiate the avalanche
process.
The Paschen curves
for some of the common discharge-tube gases are shown below. These
curves assume flat parallel electrodes of diameter comparable to or less than the length of the tube.

To give an idea of how to
interpret the graph, note that atmospheric pressure is 760 mm Hg (760
Torr, 101.325 kPa, 1.01325 bar). Therefore a vertical line
corresponding to 76 on the pressure × distance axis above
gives the breakdown voltage for a gas between a pair of flat electrodes
separated by 1 mm. For air, this gives a breakdown voltage of about 5
kV DC (3.5 kV RMS AC). Thus the air curve can (for example) be used for
working-out the voltage ratings of air variable capacitors; although a
safety margin is normally included to allow for surface roughness and
sharp edges, which can reduce the breakdown voltage considerably.
Electrodes,
incidentally, are not essential for gas breakdown. Any method that
produces a strong-enough electric field, with or without electrodes,
will also cause the gas (or regions therein) to glow; which is why
discharge tubes light up when placed near resonant radio antennas and
coils.
Once a glow discharge
between electrodes has initiated; a low resistance current-path is
formed, and passing a current sustains the discharge. Thus the tube
resistance remains low, and the glow will continue, until the current
falls below a value called the 'extinction point'. The voltage at the
extinction point is usually considerably lower than the strike voltage,
i.e., the discharge is difficult to get going, and then difficult to
stop once started.
A gas discharge tube, once it
has struck, usually has a negative resistance characteristic. This
means that the product of the voltage across the tube and the current
through it diminishes as the current is increased. Thus the current is
effectively unlimited, and must be controlled by the external circuitry
if it is not to lead to the destruction of the tube or the power
supply. Some tubes are intended to be operated in a range where the
voltage remains almost constant as the current increases (the
glow-discharge region). One such application is as a voltage regulator,
the current being limited by a series resistor in much the same way as
for a Zener diode. Tubes can also be designed to work in a range where
the voltage falls as the current increases (the arc discharge region),
and while this is often an incidental feature, such tubes can be used
to make oscillators that work in the same way as the tunnel diode
(Esaki diode) oscillator. Note that, for tubes in which the running
voltage increases as the current is reduced, the extinction voltage is
higher than the lowest running voltage.
Since the tube running voltage
is considerably lower than the strike voltage, tubes with an applied
voltage above the running voltage but below the strike voltage can be
triggered. This can occur in various ways, such as by illumination of
the cathode by ionising radiation, passage of radioactive particles
through the gas, or by the use of a triggering electrode.
When used as continuous light
sources, discharge tubes are operated using something approximating a
constant-current source. In DC circuits, this can be a ballast resistor
in series with a high voltage source, or some kind of high-voltage
inverter designed to have a high effective internal resistance (but
without the losses associated with an actual resistor). In AC circuits
at power-line frequencies, the reactance of an inductor can be used to
limit the current, but additional complexity arises from the need to
get the tube to strike and to keep it running during polarity
reversals. The original solution, as used for
neon signs before the
advent of electronic inverters, was to use a high-voltage step-up
transformer deliberately constructed to have a very large leakage
inductance (a magnetic-shunt transformer). Such a transformer is
equivalent to a an AC voltage source with a large series inductance
(but requires only a single iron core).
Lighting
tubes often
have oxide-coated thermionic cathodes, to reduce the running voltage by
enhancing the supply of free-electrons. Such cathodes are usually
heated by ion bombardment rather than by passing a current through a
resistive filament. Pre-heating of the cathode at switch-on however is
a convenient way of getting the tube to start. |
|
If
the gas pressure
is low (ca. 1% of atmospheric pressure or less), the tube emits light
at one or more characteristic wavelengths associated with transitions
between electronic states of the gas atoms or molecules. Devices with
such spiky spectra (e.g. yellow sodium-vapour street lights) are not
usable as general-purpose or photographic light-sources because they
give poor or non-existent colour rendering, but as the gas pressure is
increased, the spikes become broader. This 'pressure broadening' is a
direct consequence of the Heisenberg Uncertainty Principle, and stems
from the fact that as the pressure increases, the gas molecules or
atoms undergo
more and more collisions, thus reducing the average time for which a
molecule or atom can remain in a given electronic state. The Heisenberg
principle implies that as the lifetime of given state decreases, its
energy becomes less and less well defined, the net result being that
the characteristic emission wavelengths are smeared-out into bands. In
some (relatively) high-pressure gas discharge tubes, the characteristic
wavelengths are sufficiently smeared out that the output approximates
white light, or at least has sufficient red green and blue content to
render colours in a way that satisfies the human eye.
Another way to obtain
white light is to use the ultraviolet light from a low-pressure gas
discharge to cause phosphors to fluoresce. This, of course, is the
principle of operation of the ubiquitous fluorescent tube, which
produces UV light using a mixture of mercury vapour and argon.
Discharge tubes are
often provided with a third electrode (and sometimes a fourth). There
are two main reasons for doing so: priming and triggering. A priming
electrode is generally an additional anode or cathode connected to a
bias supply and disposed in such a way as to make it easier to get the
tube to strike. Often, in DC circuits, the priming electrode is used to
maintain a small reservoir of ionised gas (it is a sort-of pilot
light). This solves the problem that, if the maximum available voltage
barely matches the strike voltage, and the ambient background
radioactivity is low (not an entirely undesirable situation), and the
tube is in total darkness, there may be a waiting time of several
seconds (and in some cases, minutes) before the tube will strike.
As an alternative to
a priming electrode, a small amount of radioactive material can be
placed inside the bulb [see link below]. Radioactive gases such as
tritium (H
3) are often used, but the material
can also be in the form of a loose powder or some other solid mineral
inclusion. Alpha emitters are best for this purpose, since
α-particles (helium nuclei) cannot escape beyond the glass
wall. Because of the container shielding effect, the use of radioactive
material is not necessarily detectable from outside the bulb.
When a triggering
electrode is included, the tube is known as a 'thyratron' and can be
used as a controlled switch. The name of the solid state equivalent,
the 'thyristor' (aka 'silicon-controlled-rectifier') is coined from the
combination 'thyratron-transistor'. Thyratrons are made in cold-cathode
and heated cathode versions. They are by no means obsolete, and are
used in a wide range of high-voltage, high current, and fast-switching
applications. Cold-cathode thyratrons are readied for triggering by
applying, across the main electrodes, a voltage above the extinction
voltage, but less than the strike voltage. A pulse applied to the
triggering electrode then causes initially-localised ionisation that
spreads throughout the tube. Hot cathode thyratrons are essentially
thermionic triodes with gas in them, and are held-off from striking by
applying a negative bias to the grid. A fourth (priming) electrode
might also be included to promote fast and reliable triggering.
As an alternative to
an internal triggering electrode, discharge tubes can also be made to
strike by applying a high-voltage pulse to the outside of the glass
envelope. This method is typically used to trigger photographic flash
tubes, which are high-pressure Xenon arc tubes having a
pressure-broadened output spectrum approximating daylight
(high-pressure in this case being about 0.3 bar or 250 Torr). An
external priming electrode can also be used when tubes are operated
with a high-frequency AC supply (an electronic ballast), the point
being that in AC (or pulsed) operation, an external electrode can
perform the same function as an internal electrode in series with a
small capacitor.
Note incidentally, that there is an
important difference between an arc and a glow discharge. An arc
involves thermionic emission and high current-density, and does not
occur in the absence of electrodes. A glow discharge takes place in the
gas, does not require electrodes, but will progress to an arc if
electrodes are used and the current between them is allowed to rise.
Shown on the right is
a small xenon-filled discharge tube connected across a current-limited
high-voltage RF source. As the current is increased, the glow discharge
gives way to an arc. Although a still picture cannot show it, the arc
also wanders up and down between the electrodes under the influence of
convection processes in the gas. |

Glow
|

Arc
|
Structure
of the low-pressure gas discharge .
If a gas-discharge tube is connected to a high-voltage DC source, or to
an asymmetric (polarised) AC source (such as an induction coil),
various structures will be seen in the glowing column depending on the
gas pressure, the tube length, and the operating current. Not all of
the possible phenomena will necessarily be visible at any one time, but
in long tubes there will usually be found a low pressure (ca. 1% of
atmospheric pressure or less) at which judicious adjustment of the
current will cause the discharge to take on an appearance similar to
that depicted below (the glow colour however depends on the type of
gas). In some gases also (e.g., neon), the negative glow and the
positive column merge and the Faraday dark space is indistinct.
'DS'
is an abbreviation for 'dark space'.
A full explanation of the
underlying physics is not appropriate in this brief introductory
article; but the structure can be understood on the basis that there is
a current of electrons flowing towards the anode, and a current of
positive ions migrating towards the cathode. Excitation of gas
molecules to energy-states from which they emit light on falling back
to the ground-state depends on collisions with ions and electrons; and
these charged particles require a period of acceleration in the
electric field before they have sufficient energy to cause the required
collisional excitation. Also electron production occurs in waves,
starting at the cathode as a result of ion bombardment, with subsequent
secondary electron production in the gas column (and even at the anode)
as a result of collisions. Hence the various light and dark bands.
The phenomena within the tube can be separated into two distinct groups:

The cathode
glow, the negative
glow, and the Crookes and Faraday dark spaces belong to the cathode.
They are affected strongly by pressure but do not depend markedly on
tube length. The Crookes dark-space becomes longer as the pressure is
reduced, and comes to fill the entire tube as the last of the gas is
removed. The Faraday dark space is affected by the tube diameter. It is
never completely dark (there is always a diffuse glow) and it sometimes
fails to appear.

The positive
column, also known
as the 'plasma', is elongated by increasing the length of the tube. It
becomes striated because electrons are absorbed in the process of
ionising and otherwise imparting energy to the gas molecules, and newly
produced secondary electrons require acceleration before they can cause
ionisation in their turn. The interval between striations depends on
the pressure, and the visible effect is often indistinct. Striations
tend to be more obvious in polyatomic gases (O
2,
N
2, H
2O, etc.) than in
monatomic (noble) gases such as Ne and Ar. They can also give the
appearance of being stationary or moving.
Because there are two distinct sets of phenomena visible in the gas
discharge; tubes designed for light-emitting purposes are conveniently
divided into two types. These are as follows:

Cathode-glow
devices: (e.g.,
nixie tubes, indicator lamps, decorative neons, night-lights).
In cathode-glow lamps, the positive column glow is usually suppressed
by keeping the inter-electrode distance short, and the negative glow
can be suppressed by keeping the pressure low to expand the Crookes
dark space.

Positive-column
(plasma) lamps:
(e.g., fluorescent tubes, 'neon' sign tubes, flash tubes, spectrum
tubes, gas lasers).
The positive column is made prominent by using a large inter-electrode
distance. In positive column lamps, the major dark spaces and any
striations can be filled-in by using AC excitation (although judicious
choice of pressure, current and frequency is sometimes necessary to
make them disappear completely). Also, the efficiency with which
electrical energy is converted into light increases with excitation
frequency (up to a limit), which is why modern compact fluorescent
lamps (CFLs) and neon-sign lamps are operated at several 10s of kHz.
For a given gas, the glow colour obtained in a cathode-glow lamp can be
radically different from that obtained in the positive column. This is
especially the case when a mixture of gases is used. In cathode-glow
lamps, the positive glow is not normally seen, but it can be excited by
placing the tube in a strong radio-frequency field. Comparison of the
two glows can then reveal the gas mixture. In some nixie tubes for
example (see
XN12),
a mixture of neon and mercury vapour is used. In this case the cathode
glow is orange, but the positive glow is blue-green (i.e., cyan). Haze,
due to incomplete suppression of the positive glow, can in this case be
eliminated by the use of a red filter (the tubes are often dipped in
red varnish).
Discharge
tube: current vs. voltage characteristic .
Shown below is a 'typical' current vs. voltage characteristic for a gas
discharge tube. The graph is only typical however in the sense that all
tubes exhibit the conduction behaviour shown, but the corresponding
voltages and currents, and the relative lengths of the various regions,
are highly variable. The axes labelled as shown might correspond
roughly to the characteristic of a voltage-regulator tube without a
priming electrode. The characteristic is obtained by operating the tube
from a variable-voltage power supply with a series (ballast) resistance.
Note, incidentally,
that the graph is easiest to interpret if it is borne in mind that the
voltage is the independent variable, and the current is the measured
quantity; i.e., the curve is what happens as we turn the voltage up.
Also note that the graph shows transitional events that cannot be
reversed by turning the voltage down; i.e.; the curve has hysteresis.
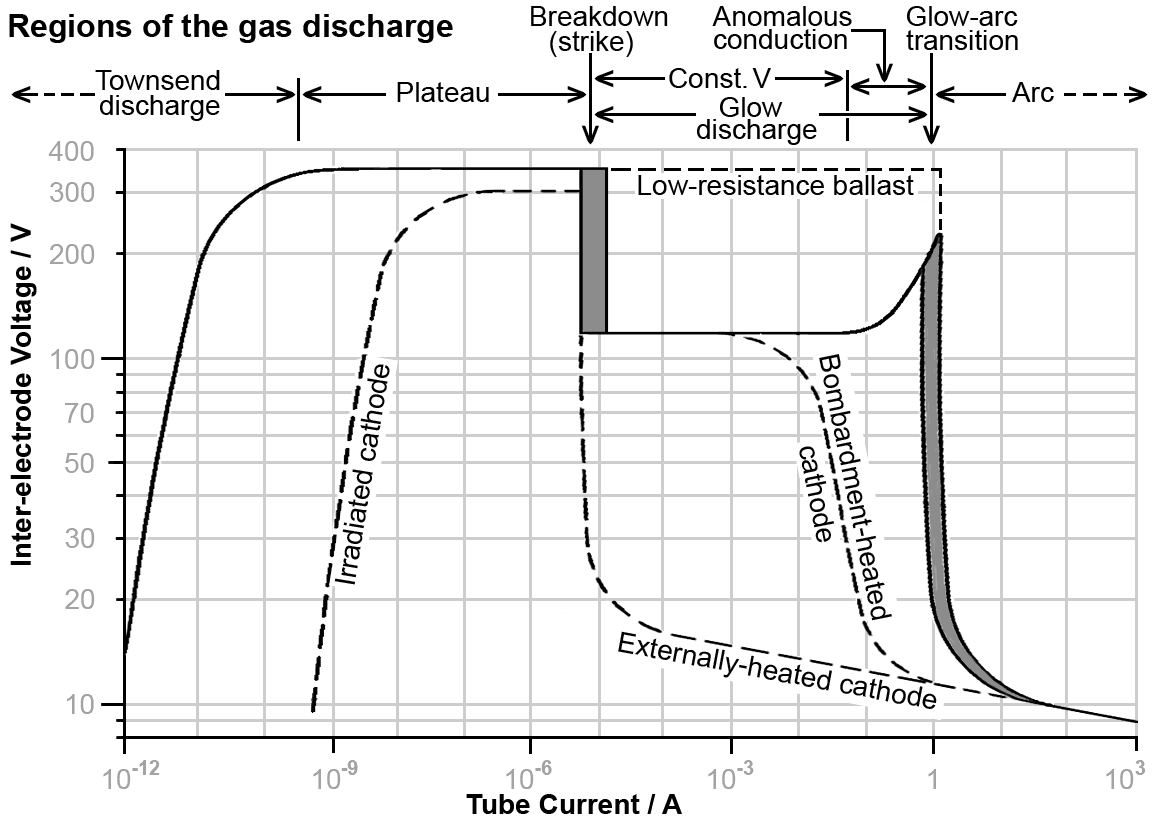
There is some conduction through
the tube before it reaches its breakdown or 'strike' point. This is
known as the 'Townsend discharge', or 'dark discharge', and is due to
ionisation caused by collisions between gas molecules. Increasing the
inter-electrode voltage will lead to a point where the current
increases dramatically for a very small increase in voltage, and hence
a voltage plateau is reached..
Operation in the
plateau region is unstable. This is because the electric field is
strong-enough to accelerate free electrons to a degree that allows them
to ionise gas molecules and liberate more electrons. When the current
reaches a critical point, an avalanche effect occurs and the discharge
becomes self-sustaining. This is the breakdown point, at which the gas
starts to glow, its conductivity suddenly increases, the voltage-drop
across the ballast resistance correspondingly increases, and the
inter-electrode voltage drops abruptly. The breakdown point is noisy
and ill-defined , and so is shown in the graph to be likely to occur
over a range of currents. It is also uncertain in time, requiring a
random fluctuation to set it off, and so may be delayed for several
seconds (or even minutes).
The highest possible
plateau voltage prior to striking will not necessarily be reached. If
the cathode is illuminated by any ionising EM radiation, or if there
are any radioactive events affecting the gas or the cathode, then the
tube will strike early. If the tube is kept well away from the
breakdown point however, the increase in current due to irradiation is
reversible and allows the tube to be used as a radiation detector.
If there is a
relatively-high series resistance, the tube will enter the glow
discharge region upon striking. If the gas pressure is relatively high,
this is characterised by a cathode-glow covering only part of the
cathode, and a running voltage that remains nearly constant over a wide
range of currents (at least an order-of-magnitude, often more). The
glow tends to concentrate at the part of the cathode having the lowest
electron-work-function. The rate of electron production is limited
however, and so as the current is increased, the glow coverage
increases. Often, also, the glow patch is unstable and moves around on
the cathode. This is because the gas is not in thermal equilibrium. The
hot region around the glow patch reduces the gas density and causes the
point of lowest running voltage to move to a new location. Thus the
glow tends to flicker, but the phenomenon causes fluctuations only of
the order of a few mV in the running voltage, and so does not affect
the utility of the tube as a voltage regulator. The flickering effect
can be deliberately enhanced however (for
novelty flame-effect lamps,
etc.) by using special cathode coatings to obtain a uniform
work-function.
At the end of the
constant voltage (CV) region, comes the point at which the cathode glow
coverage is complete. This leads to a region in which further increase
in current is accompanied by an increase in running voltage. This, of
course, is normal behaviour for ordinary conductors; but gas discharge
tubes are regarded as negative-resistance devices, and so this is known
as the 'anomalous conduction' or 'abnormal conduction' region. The
useful current-range of this region can be increased by keeping the
cathode area small and making the gas pressure relatively low (at some
cost in terms of light output). Cathode glow lamps that must produce a
stable display (such as nixie tubes) are operated in this way. Also,
tubes that must quench in response to a reduction in applied voltage
(such as glow-switches) are optimised for this region.
At the end of the
abnormal conduction region there is another abrupt transition and an
arc discharge begins. This is characterised by very high current
density and an inter-electrode voltage that falls as the current is
increased. The surface electrode temperature is equal to the boiling
temperature of the electrode-material or coating, and electrodes must
be made of extremely refractory material if they are not to be rapidly
consumed. Once again, the current at the onset-point is uncertain.
Note that the
existence of a glow-discharge region prior to the onset of
arc-conduction is a matter of choice of ballast resistance. If the
resistance is low, the tube will pass straight into arc-conduction mode
after striking.
A direct transition
to an arc-like conduction mode is also obtained in tubes with heated
cathodes. The electrical characteristic is that of an arc commencing at
an unusually-low current, but whether it should be referred to as an
arc is moot. Strictly, an arc is self-sustaining, whereas this type of
conduction will change into a glow-discharge if the heater-supply is
turned off.
Tubes with thermionic
cathodes heated by ion bombardment (fluorescent tubes, etc.) exhibit a
variant of arc-like-conduction onset if started without the use of
cathode pre-heating. The discharge begins as a glow-discharge, but goes
into arc-like conduction mode as soon at the cathode reaches working
temperature. For such tubes, there is no anomalous conduction region.
Operating region for various
types of discharge
tube
Tube
oscillations .
Most gas discharge tubes, being negative-resistance devices, tend to
oscillate. A simple oscillator can be made, for example, by operating
the tube via a ballast resistor and placing a capacitor directly across
it. In this case, the ballast resistor charges the capacitor until the
strike voltage is reached. Then, assuming that the capacitor has a low
internal resistance, the tube goes into arc-conduction mode until the
extinction point is reached. The capacitor then recharges and the cycle
repeats. This type of circuit is known as a 'relaxation oscillator'.
Relaxation
oscillators normaly run at low frequencies, but with some tubes, it is
possible to get the circuit to work at several 10s of MHz. The
oscillations so produced also tend to have a very large large noise
bandwidth, and can be deleterious to radio-signal reception. Hence it
is essential that tubes used in radio systems have very low shunt
capacitance; this being an important consideration when using nixie
tubes in frequency-readout circuits. The basic precaution is to place
the ballast resistor directly adjacent to the anode connection, and
give this part of the circuit plenty of room on the circuit board.
A more sophisticated
negative-resistance oscillator can be made by feeding a DC arc via a
choke and placing a series-resonant LC circuit across the tube. If the
arc can change its conduction characteristic fast-enough to respond the
the resonant oscillation of the LC network, the negative-resistance
cancels the losses in the resonator and regenaritive oscillation
occurs. This method was used to produce continuous-wave radio signals
before the advent of the hard-vacuum triode oscillator (see, for
example: '
Alternator Arc and Spark',
by Duncan Cadd); although a very fast-quenching arc (hydrogen
atmosphere) is required if this is to work at long-wave radio
frequencies.
Further information and
bibliography:
Electron
Tube Design,
RCA 1962 (complete book available from:
tubebooks.org). See section:
'Gas
Tube Design' by H H Wittenberg, p792 - 817.
Low-pressure gas breakdown in a
uniform dc
electric field. V A Lisovskiy, S D Yakovin
& V Yegorenkov. J Phys. D. Appl. Phys. 33 (2000), p2722-2730.
[Paschen's law is not strictly true. There is also a dependence on
vessel length/radius ratio. A method of predicting breakdown in
cylindrical vessels of arbitrary L/r, using existing data, is given).
Conduction of electricity through gases,
J J Thomson. 2nd edition 1906 (internet archive). Ch 16, p527.
(3rd edition, 1928, also exists, out of copyright since 2010 but
currently not available free).
The Passage of Electricity
Through Discharge Tubes at Low Pressures.
R Whiddington.
Science Progress in the 20
th Century
(1919-1933). Vol 20, No, 80, April 1926, pp 620-636
Available from
Jstor.
Interesting discussion of striations (beading) from p633 on.
Conduction
of
Electricity Through Gases, J Benyon. Harrap 1972.
Physical
Electronics,
C L Hemmingway, R W Henry, M Caulton, Wiley, 2
nd
edn. 1967.
Basic
Processes of
Gaseous Electronics, L B Loeb, U Cal. 2
nd
edn.1961.
Gaseous
Conductors,
Theory and Engineering Applications, J D Cobine, Dover 1958
(corrected version of 1941 edition).
The
Development of
Gas Discharge Tubes. J D Cobine. Proc. IRE, 50(5), 1962.
p970-978.
Historical review of gas discharge tube development. Tubes are
clasified into 5 groups: (1) Ionization tubes, (2) Cold-cathode
discharge tubes, (3) Hot-cathode arc discharge tubes, (4) Liquid metal
arc tubes, and (5) Plasma tubes.
Ions,
Electroms and
Ionizing Radiations, J A Crowther, Arnold. 7
th
edn. 1938.
Principles
of Electricity and
Electromagnetism, G P Harnwell, 1938.
Radio-Frequency
Capacitive Discharges. Y P Raizer, M N Schneider, N A
Yatsenko, CRC Press, 1995.
(Thanks to
Dr Duncan Cadd (G0UTY) for the
donation of this book).
Engineering
Electronics, G E Happell, W M Hesselberth. McGraw-Hill 1953.
LCCN 53-5166 (Available from:
tubebooks.org). See Ch. 13.
Conduction through gases, p385 - 407.
Electric
Breakdown in Long Discharge Tubes at Low Pressure
(Review, Oct. 2020, Open Access).
Yu Z Ionikh.
https://rdcu.be/cnR5z

A
List of 'valves' (i.e., discharge
tubes) containing radioactive elements. is available from
RoyalSignals.org
.